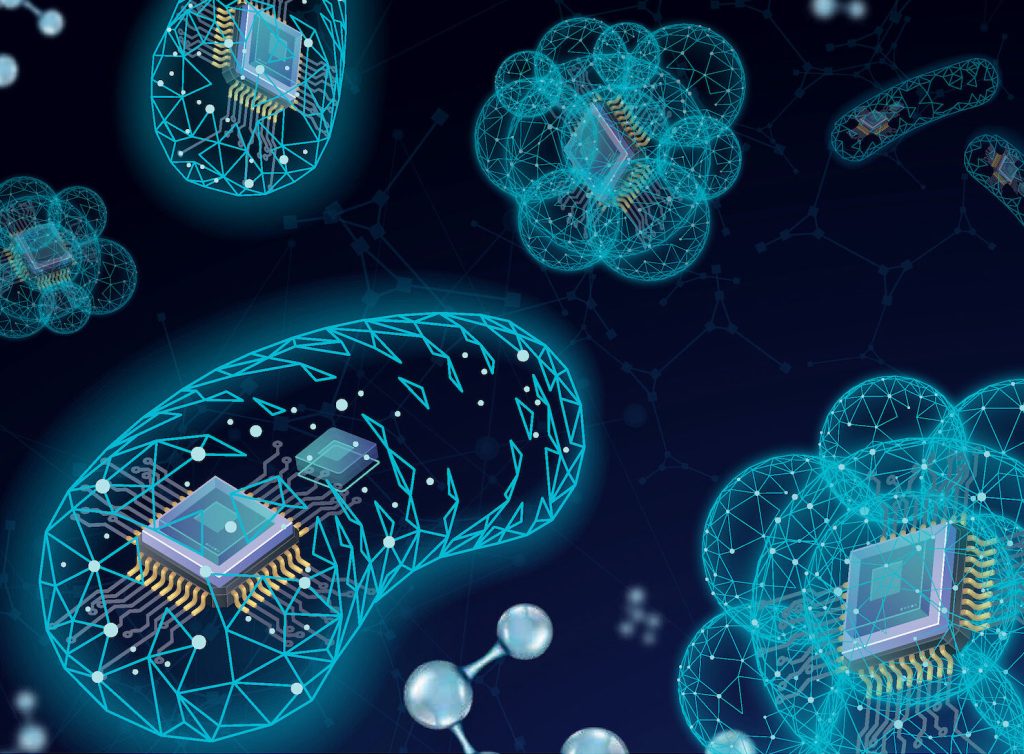
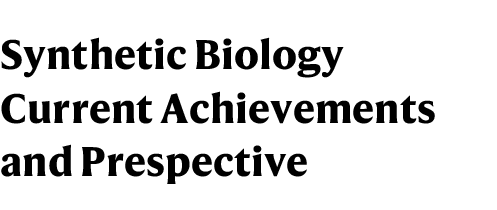
Home » Trends in Biotechnology » Synthetic Biology: Current Achievements and Prespective
Synthetic biology is a rapidly emerging field encompassing the design and construction of novel biological pathways within microorganisms, as well as the redesign of natural biological systems.
The impact of this technology is poised to revolutionize our methods of food production, reshape our dietary choices, and transform the sources of our raw materials and medications. In this article, we present a selection of products that have already been created using synthetic biology and are currently available in the market. We delve into the underlying technologies that have made these innovations possible and offer insights into the promising future that awaits us in the coming decade.
Early research in the realms of cell design and the physical structure of DNA encountered significant challenges and instability prior to 2010. However, notable breakthroughs in areas such as metabolic engineering, directed evolution (awarded the Nobel Prize in 2018), strain engineering, metagenomics discovery, genetic circuit design, and genome editing (awarded the Nobel Prize in 2020) have made synthetic biology not only feasible but also increasingly sophisticated.
Contents
Synthetic Biology in The Food Industry
In 2019, the first oil produced using synthetic biology was introduced, marking the entry of an engineered plant-derived product into American food sources. Soybean oil, which constitutes 90% of seed oil, is known for its high content of unstable linoleic acid that degrades rapidly when exposed to heat. To address this issue and reduce food waste, scientists have edited the soybean genome by deactivating two genes responsible for fatty acid saturation. As a result, the modified soybean produces an oil containing 80% oleic acid, compared to the unmodified soybean’s 20%. This modification provides a more stable oil option while retaining the benefits of soybean oil.
Synthetic biology has also played a role in the development of plant-based burgers that closely resemble the taste and texture of beef. By incorporating meat-like additives derived from engineered yeasts using leghemoglobin, these hybrid burgers require less agricultural land and produce minimal greenhouse gases compared to traditional beef burgers. These innovative products can be found in over 30,000 restaurants and 15,000 grocery stores worldwide.
Gene editing techniques, such as transcription activator-like effector nucleases (TALENs) and RNA interference (RNAi), have been pivotal in achieving precise modifications in the genome. However, the design of these techniques can be challenging. The emergence of CRISPR/Cas9 has addressed this issue, allowing for the easier design of guide RNAs that direct the Cas9 nuclease to specific targets, enabling DNA replacement, deletion, or addition. This powerful tool has facilitated the production of many genetically edited products that enhance yield and nutritional value, address contamination and pests, and exhibit adaptability to various conditions.
Examples of genome-edited food products include tastier mustard plants and higher-yielding blue corn, which entered the food sources in 2021 using CRISPR/Cas9. Other genome edits have been applied to beef, chicken, fish, sheep with longer wool, goats producing human whey protein in their milk, virus-resistant pigs, and chickens laying allergen-free eggs.
Advancements in metabolic engineering have made it easier to transfer complex metabolic pathways from natural sources to production hosts like yeast. This has been made possible through the use of computational tools for pathway design, enzyme engineering techniques, precise gene libraries, and genome editing for pathway modification. Engineered yeasts are now capable of producing a wide range of food additives, including vitamins and milk proteins.
Bioinformatics and DNA synthesis techniques are also utilized to transfer metabolic pathways from plants or microorganisms to production hosts for screening and identifying potential drugs. This enables access to chemical compounds that are scarce in nature by transferring the pathways to prokaryotic and eukaryotic hosts.
Synthetic Biology in Electronic Systems
Hyaline is a transparent, flexible, and mechanically strong polyimide layer derived from bio-based monomers. This unique family of layers has found applications in flexible electronic devices such as foldable smartphones and wearable gadgets, with examples of these products being introduced in early 2021. While polyimides, including the well-known Kapton, are renowned for their chemical-thermal stability and superior mechanical properties, their inherent color often restricts their use in applications requiring transparency. However, hyaline layers overcome this limitation by utilizing diamine monomers produced using engineered organisms in conjunction with artificial intelligence. Through the use of robotic tools, millions of strains can be simultaneously generated, facilitating the genetic reprogramming of cells to create a new material. Synthetic biology’s initial foray into materials production involved the development of small carbon-based molecules as cleaner substitutes for petroleum derivatives, such as ethanol, propanediol, or butanediol.
In addition to novel polyimides, the design of protein materials has been simplified to exhibit desirable properties. For instance, elastin-silk hybrids combine thermal resistance with flexibility. Efforts are now underway to transfer this capability to other polymers like polyesters through ribosome engineering. Furthermore, by manipulating polymers into nanostructures, biology can control their optical or electrical properties. Melanin, for example, assumes various functions such as UV protection, luminescent material, or light-generating cells (similar to fireflies) based on its specific shape. Synthetic biology extends beyond carbon-based materials and can be employed to create materials containing at least 55 elements, including rare earth minerals and uranium. Through the use of engineered reducing enzymes and phage display techniques, mineral-based nanomaterials for lightweight batteries, catalysts, solar cells, and optics have been successfully produced.
Synthetic Biology in Agriculture
In agricultural practices, the supplementation of nitrogen in soils is crucial for achieving high crop yields. However, the current methods predominantly rely on energy-intensive chemical processes, accounting for approximately 1-2% of global energy consumption. While biological nitrogen fixation bacteria are used as natural nitrogen fertilizers, they are not compatible with cereal crops such as corn. To address this limitation, researchers have developed the first biofertilizer specifically designed for corn cultivation. This biofertilizer establishes a symbiotic relationship with corn roots and possesses the necessary genes for nitrogen fixation. Although these genes are naturally dormant, synthetic biology techniques have been employed to activate them. The biofertilizer, which contains this bacterium as its active ingredient, is in liquid form and has shown the potential to reduce the requirement for chemical fertilizers by up to 12 kilograms per hectare while increasing yields by up to 5.8 times. Importantly, unlike chemical fertilizers, this biofertilizer does not leach nitrogen into groundwater or release nitrogen as N2O, a potent greenhouse gas, into the atmosphere.
The adoption of this bio-based product has been substantial, with its usage expanding from 250,000 hectares of agricultural land in 2020 to millions of hectares in 2021. Microbial communities in the environment possess the potential to contribute beneficial applications to ecosystems and eliminate harmful functions. However, due to the complexity and lack of comprehensive characterization of microbial environments, harnessing their potential can be challenging. Advances in microfluidics, synthetic biology, and genome editing have facilitated the integration of large DNA fragments, making it easier to manipulate microbial communities. Theoretical principles from control theory and virtual machines can be employed to design genetic systems that do not require extensive reconstruction when implementing them in alternative frameworks. Using these techniques, researchers are exploring and testing engineered bacteria associated with plants to increase agricultural yields, provide protection against pests, and enhance tolerance to a broader range of water, climate, and soil conditions.
Synthetic Biology in Medical and Pharmaceutical Science
The therapeutic use of genetically engineered living cells, known as the “third pillar of medical science,” has gained significant attention. CAR-T cells, a notable example, are generated by isolating T cells from patients, genetically modifying them to express a chimeric antigen receptor (CAR), and then reintroducing them into the patient’s body. These engineered cells can exhibit long-term stability, lasting for several years or even decades. Remarkable improvements, reaching up to 83%, have been observed in patients with relapsed or refractory diseases following CAR-T cell therapy. Pharmaceutical agents based on this approach have entered experimental investigation and production, primarily targeting hematological malignancies since the summer of 2020. However, there is a growing interest in expanding their application to include solid tumors, autoimmune disorders, and viral infections.
The successful development of effective living therapeutics requires a deep understanding of synthetic regulatory pathways, particularly genetic circuits. Introducing a specific antigenic target can inadvertently induce toxicity in non-target cells, and mutations in the targeted antigen can lead to drug resistance. To overcome these challenges, intricate gene circuits have been designed to incorporate multiple sensor inputs. Some genetic circuits with AND gates have been implemented to enhance selectivity, while others with OR gates help to circumvent resistance phenomena. Notably, the activities of CAR-T cells can be precisely controlled in time and space by utilizing biologically derived sensors. In cases where patients experience cytokine release syndrome, a common adverse effect, safeguard switches have been developed to enable rapid attenuation of CAR-T cell activity. Genetic circuits are highly responsive to changes in gene expression levels that may occur due to stochastic lentiviral integration. This issue has been addressed by leveraging genome editing techniques to introduce specific “landing sites” that bypass this problem.
Beyond T cells, genetic circuits and genome editing methodologies have become essential tools for exerting precise control over the localization and temporal dynamics of therapeutic living cells. Furthermore, genome editing is being applied to the development of human drug therapies, including the production of safer and more effective CAR-T therapies, novel gene delivery systems (such as clinical trials for treating hereditary ocular disorders), DNA-based identification systems for in vitro cancer diagnosis and pathogen detection, and improved approaches to organ transplantation that prioritize enhanced patient well-being.
Synthetic Biology and the Future
An animated depiction of a future where people fly in helicopter-like drones, houses are shaped like trees, and living spaceships resemble fish presents an imaginative portrayal of the integration of biological elements into society. One can envision NASA utilizing synthetic biology to produce food and medicine during long-duration space missions. These products mentioned already generate a combined annual revenue of two billion dollars and are expected to further increase their market share outside of the pharmaceutical industry. In order to fully grasp and interpret this vision in 2030, one would need to examine hundreds, if not thousands, of products that will emerge from the ongoing wave of innovation.
As the global population continues to grow and more products are derived from fermentation, there will be a decrease in the use of sugar in the production of consumer goods. In the coming decades, it will become crucial to establish new microbial frameworks that can produce carbon from alternative sources, such as plastic waste or atmospheric carbon dioxide, either directly or through non-organic coupling to “artificial leaves.” Additionally, freshwater, a limited resource with significant applications in fermentation, can be addressed by designing salt-tolerant models that can grow in bioreactors using seawater.
Beyond 2030, products are likely to evolve into systems where cells are designed to work together or be integrated into inert or electronic materials. In the field of agriculture, genetically engineered plants may receive information from drones through collaboration with bacteria, and in turn, send signals to control gene expression in response. In the future, hamburgers could be produced by a consortium of bacteria, fungi, and cells working together to create molecules that enhance nutritional value, taste, and aroma. Building materials could be enhanced by engineered living cells that exhibit functions like self-healing or air pollution remediation, resembling the living structures of Singapore. Engineered organisms could be utilized to prevent biofouling on ship hulls, reduce pipe corrosion, or spray on soil to stabilize airport runways. The integration of engineered living cells with electronic materials would enable the development of interfaces between the brain and computers, as well as the creation of robots utilizing biological sensors for navigation or self-powering. However, achieving these ideas in reality requires the development of design tools that are highly reliable, making screening and modeling methods unnecessary, and allowing for the measurement of results in simulated environments that closely resemble the real world.